Autonomic Cardiovascular Control in Health and Disease
- Dr Luigi Vorluni
- Feb 27
- 17 min read
Updated: May 7
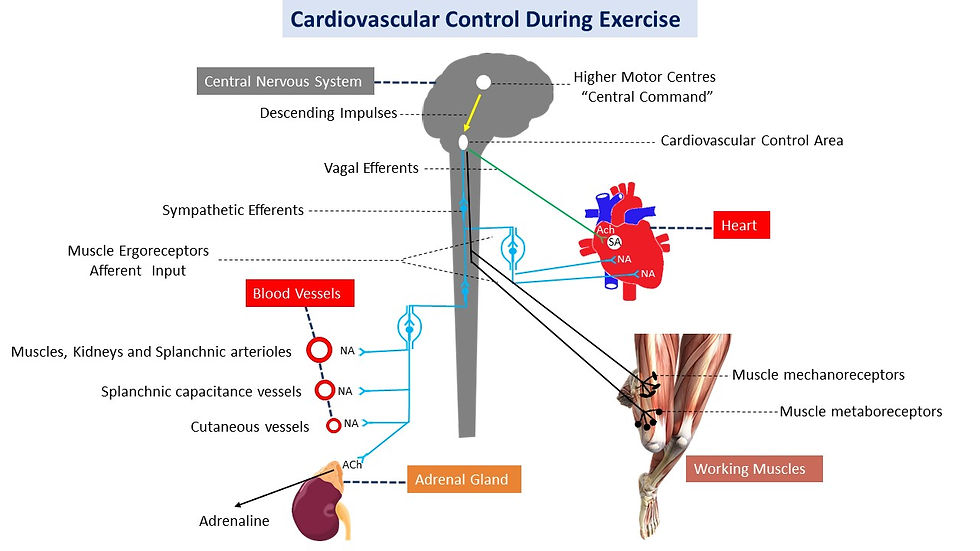
Abstract
Homeostasis of hemodynamics refers to the control of circulation in order to meet the ever changing metabolic demand of the different tissues and maintain normal physiological functions, by distributing cardiac output and blood flow optimally. The dynamic cardiovascular control results from complex interactions between various neural circuits, including central command, arterial baroreflex, mechanoreflex, metaboreflex and autonomic nervous system. Cardiovascular diseases are a major cause of morbidity and mortality in many countries worldwide. Parasympathetic and sympathetic traffic plays a key role in cardiovascular control and health. Reduced cardiac vagal control is associated with poor cardiorespiratory fitness, increased risk of adverse cardiac events and insulin resistance. Performing physical exercise regularly brings about various health benefits, such as improved cardiac electrical stability and decreased risk of adverse cardiac events. Therefore, exercise should be incorporated within the interventions in cardiology to tackle poor cardiovascular fitness and help tackle cardiovascular diseases.
Introduction
The primary role of the cardiovascular control mechanisms is to maintain homeostasis, adequate arterial pressure and tissue perfusion (Alam et al., 1937, Rowell, 1993). Homeostasis of hemodynamics refers to a moment-to-moment regulation of the blood circulation to meet the demand of the different tissues and organs (De Hert, 2012).
Maintenance or prompt restoration of blood perfusion, oxygen and nutrients supply in sufficient amount to meet cellular metabolism, is fundamental for sustaining normal physiological functions (Parker et al., 2019). This is particularly relevant when the metabolic needs change significantly, for example in response to increased physical activity (Peterson et al., 1988).
Because the vasodilation ability is greater than the capability of increasing cardiac output, thus there is not sufficient blood flow to distribute blood equally to all tissues simultaneously and consequently, the cardiovascular system must be regulated for resource allocation, For instance, during physical exercise, more blood must be directed to the working muscles, the heart, and the lungs. While in a postprandial state, more blood is directed to the digestive system. Only blood perfusion of the brain is maintained more or less constant (Blomqvist et al., 1983, Anderson et al., 1985, Peterson et al., 1988, Powers et al., 2012).
The dynamic circulatory control results from complex interactions between various neural circuits, including central command, arterial baroreflex, mechanoreflex, metaboreflex and autonomic nervous system (ANS, Andres et al., 1985, Thimm et al., 1987, Rowell, 1993, Adreani et al., 1997, Raven et al., 2016). This article focuses mainly on ANS control of circulation and its importance for fitness and health. Interventions to improve cardiac vagal control are also discussed.
The autonomic nervous system
The ANS is separated both structurally and functionally into two divisions, the parasympathetic nervous system (PNS), and the sympathetic nervous system (SNS, Shields, 1993). Parasympathetic and sympathetic outflow to the various tissues and organs changes constantly in response to various stimuli. Typically when parasympathetic traffic increases, sympathetic traffic decreases, and vice versa. These changes in sympathovagal balance have profound effects on the function of numerous organs (Kiernan and Rajakumar, 2014). The ANS play a key role in cardiovascular control, and represents the efferent branch of the arterial baroreflex. The ANS contributes to the maintenance of circulatory homeostasis by regulating the functions of the heart and smooth muscles in the blood vessels, and hormone secretion (Ravits, 1997).
Parasympathetic nervous system
The PNS consists of relatively long preganglionic cell bodies, located principally in the nucleus ambiguus (NA, Gunn et al., 1968) and dorsal motor nucleus of vagus (DMNV, McAllen and Spyer, 1976). The populations of the efferent preganglionic PNS (X nerve) neurons are situated in the medulla oblongata (Wang et al., 2003b), and project their axons via the vagi to intrinsic cardiac parasympathetic efferent postganglionic neurons. In turn, the postganglionic neurons innervate the sinus node, the atrioventricular conduction pathway and the atrial myocardium (Furnival et al., 1973, Hainsworth, 1995, McAllen and Spyer, 1978), while there is increasing evidence for the existence of ventricular vagal innervations as well (Standish et al., 1994, Johnson et al., 2004). Canine studies indicate that the intrinsic cardiac nerves of the ventricles consist primarily of postganglionic parasympathetic axons, which arise from supraventricular ganglia and cross the atrioventricular groove (Blomquist et al., 1987). The NA is very important for the control of circulation, as destruction of the NA causes degeneration of cardiac vagal fibres (Szentagothai, 1964) and abolishes baroreceptor-cardiac responses (Chen et al., 1972).
Stimulation of the preganglionic parasympathetic nerve releases the neurotransmitter acetylcholine (ACh) at the ganglion, which acts on nicotinic receptors of the postganglionic nerve (Vanhoutte et al., 1980). The short postganglionic nerve endings then release ACh to stimulate the muscarinic receptors of the target organ (Levy et al., 1970, Calaresu et al., 1975). The cardiac parasympathetic effect occurs principally through the binding of ACh to the postganglionic M2 muscarinic receptors of the cardiac sinoatrial and atrioventricular node. Subsequently, a rapid (within 100ms) membrane hyperpolarization and attenuation of both the conduction velocity of the atrioventricular node (AV node) and the contractility of the cardiac muscle, induce cardiodeceleration and decrease in cardiac output (Q) within 0.4 second (s, O'Leary, 1996). Following the cessation of vagal nerves discharge, the effect dissipates rapidly as ACh is quickly hydrolysed (Guyenet et al., 1995).
The cardiac vagal activity is an important indicator of health and fitness, as it is diminished and unresponsive in many disease states, while restoration of parasympathetic activity to the heart lessens ischemia and arrhythmias and decreases the risk of sudden death (Wang et al., 2003a, Wang et al., 2003b). Furthermore, delayed decrease in heart rate (HR) during the first minute after graded exercise, which may be a reflection of decreased cardiac vagal activity, is a powerful predictor of overall mortality, independent of workload, the presence or absence of myocardial perfusion defects, and changes in HR during exercise (Cole et al., 1999). In addition, cardiac vagal activity is capable of preventing ventricular arrhythmias by maintaining cardiac electrostability during high cardiac sympathetic outflow, often observed in patients with cardiovascular diseases (Billman, 2002).
Sympathetic nervous system
Neurons in the caudal and rostral ventrolateral medulla (VLM) synapse with sympathetic preganglionic neurones in the intermediolateral nucleus of the spinal cord (Barman and Gebber, 1985), and are important sites for the control of sympathetic outflow (Reis and Cuenod, 1965, Willette et al., 1984b, Ciriello et al., 1986), and thus the baroreflex control of circulation (Bauer et al., 1989, Guyenet and Koshiya, 1995). Electrical stimulation of the VLM provokes large increases in blood pressure (BP) and HR (Dampney and Moon, 1980, Ross, 1983) while destruction of this area, or injection of inhibitory aminoacids, leads to profound hypotension (Dampney and Moon, 1980, Ross et al., 1984). The VLM units display two types of discharge patterns in response to muscle contraction; a rapid onset response occurring within 3-5 s and a delayed onset response occurring within 10-20 s (Bauer et al., 1989). Additionally, 14 of 28 VLM units tested had a cardiac-related rhythm and 10 of those 14 also responded to muscular contraction (Bauer et al., 1989). Furthermore, muscular contraction has no effect on the discharge patterns of most neurons located outside the VLM (Bauer et al., 1989). Collectively, these studies provide evidence of the important role of VLM in the control of circulation.
All preganglionic neurons of the SNS are located in the spinal cord. The majority of cardiovascular sympathetic nuclei are situated in the lateral horn of the gray matter at the T1-L4 of the spinal cord called intermediolateral cell column (Calaresu et al., 1975). The sympathetic preganglionic neurones are relatively short, exhibiting myelinated axons, and synapse with the paravertebral ganglia located laterally to the spinal cord and form the truncus sympathicus (Barman and Wurster, 1975). These sympathetic preganglionic fibres then leave the spinal nerve trunk and travel to the ganglia of the sympathetic chain, particularly to the stellate ganglia, where they synapse with the post ganglionic fibres. The sympathetic axons of the postganglionic neurons are relatively long and unmyelinated, and travel to the target organs (Gebber et al., 1997). From the target organs, axons travel back to the spinal cord and part of them travel on to the skin and muscles through the spinal nerves (Barman and Wurster, 1975). Sympathetic innervation of the heart arises from the cervical and upper thoracic (stellate) ganglia. In general, excitatory (e.g. L-glutamate) and inhibitory (e.g. GABA) substances mediate rapid communication within the central autonomic circuits e.g. baroreflex pathways (Sun and Guyenet, 1985).
The cardiac sympathetic fibres are distributed to all parts of the heart with higher number in the ventricles (Dampney, 1994). The principal neurotransmitter for preganglionic sympathetic neurones is ACh (Feldberg and Gaddum, 1934), while the principal post ganglionic neurotransmitter is noradrenaline (Bolme and Fuxe, 1970). The sympathetic control of blood vessels diameter occurs via binding of noradrenaline and adrenaline to postganglionic α- and β-adrenoreceptors; while cardioacceleration as well as the increase in contractility occurs mainly via the catecholamine induced stimulation of the cardiac β1-adrenoreceptors (Bolme and Fuxe, 1970). Cardiac responses to sympathetic stimulation arise relatively slowly (2-5 s, Levy, 1984). This is caused by the slow dispersion of noradrenaline following its release at sympathetic nerve endings; nonetheless, within about 30 s steady state is reached (Levy, 1971). The effect of sympathetic stimulation dissipates more gradually than the onset phase, due to a slow noradrenaline reuptake by sympathetic nerve terminals, and slow rate of noradrenaline metabolism by the cardiac tissue (Irisawa et al., 1993).
Sympathetic and parasympathetic interaction in the sinoatrial node
The heart is constantly under autonomic control. Vagal stimulation tends to decrease cardiac contractility and HR, while sympathoexcitation tends to increase these cardiac indexes. Interaction between the SNS and PNS in HR regulation occurs through prejunctional and postjunctianal mechanisms (Rosemblueth and Simeone, 1934 ). In the postjunctional mechanism, ACh is released from vagal nerve terminals causing an outward K+-current in the sinoatrial (SA) node cells, which may reduce the depolarization rate of the cells, and thus the discharge rate (Osterrieder et al., 1980, Sakmann et al., 1983). Additionally, ACh decreases the Ca++ current and this affects the rate of depolarization as well (Sunagawa et al., 1998). In contrast, noradrenaline has opposite effect, as it augments both the Ca++ current and depolarization rate. However, this effect is inhibited by ACh in low concentrations (50-100 fold lower for direct K+, or Ca++ current effect, Giles and Shimoni, 1989), via attenuation in the rise of intracellular ions concentrations (Rosemblueth and Simeone, 1934 ). In the sympathovagal prejunctional interactions, ACh released by vagal stimulation inhibits the release of noradrenaline by acting on the receptors of the sympathetic nerve terminals (Levy and Zieske, 1969, Levy, 1984).
On the other hand, sympathetic stimulation inhibits ACh release by acting on the receptors of the parasympathetic nerve terminals (Sunagawa et al., 1998). However, these cardiac sympathovagal interactions are more complex and not simply mutually inhibitory. This view is supported by Levy, (1984) who reported that the level of vagal activity exerts a more prominent effect in the presence of substantial sympathetic activity than vagal activity alone. This is consistent with a previous study (Levy, 1971) where concurrent sympathetic activation exaggerated the effect of cardiac vagal stimulation, due, at least in part, to cyclic AMP accumulation (Sunagawa et al., 1998). This phenomenon is termed accentuated antagonism, implying that the effects of sympathovagal stimulation on HR are not simply additive. Furthermore, studies in rabbits indicate that the cardioacceleration due to adrenaline is suppressed by ACh, and the cardiodeceleration due to ACh is enhanced by adrenaline (Mackaay et al., 1980). Therefore, this functional inhomogeneity of the sinus node explains the predominant effect of ACh over that of adrenaline (Mackaay et al., 1980). In addition, changes in vagal impulse frequency affects HR about 5-10 times faster than changes in sympathetic pulse frequency (Levy, 1971). This is due to a faster vagal pathway transmission, and more rapid synaptic ACh release and re-uptake than noradrenaline (Warner and Cox, 1962, Borst and Karemaker, 1983). Taken together these studies clearly indicate that the cardiac sympathovagal interactions are rather complex, and that the vagal activity exhibits a more predominant and rapid action on HR, compared with sympathetic activity.
Autonomic cardiovascular control, physical exercise and health
Cardiovascular diseases (CVD) are increasing at an alarming rate and remain a major cause of morbidity, premature mortality and rising health care costs (Mensah et al., 2019, Murray et al., 2020, Vos et al., 2020). Unless appropriate countermeasures are implemented the trend is set to continue, impacting on individuals, communities and society. Heart rate variability (HRV) is a non-invasive method to evaluate autonomic cardiovascular control (Task Force of the European Society of Cardiology and the North American Society of Pacing and Electrophysiology, 1996). Research has demonstrated that decreased HRV is the result of reduced reflex cardiac vagal control (Barron and Lesh, 1996).
Reduced HRV is associated with greater risk for ventricular fibrillation during transient ischemic episodes that occur after myocardial infarction (MI, Schwartz et al., 1988, Hull et al., 1990, Billman et al., 1982), low cardiorespiratory fitness, and other risks factors of CVD (Odemuyiwa et al., 1991), and insulin resistance (Pober et al., 2004).
Moreover, reduced HRV may be predictive of a second MI and an independent predictor of risk for sudden death in asymptomatic individuals (Buccelletti et al., 2009, Molgaard et al., 1991). Moreover, there is evidence suggesting that HRV is also a correlate of stress (Berntson et al., 2007). Interventions that improve HRV can decrease the risk of adverse cardiovascular events (Parati et al., 2000). It has been shown that exercise training can improve HRV and cardiac vagal control in patients with coronary artery disease (Pagani et al., 1988, Somers et al., 1991). In addition, physical exercise may improve cardiac sympathovagal balance (Iellamo et al., 2000), cardiac electrical stability, and prevent ventricular fibrillation (Pober et al., 2004, Gulli et al., 2003, Billman et al., 1984, Hull et al., 1994, Ueno et al., 2002, Farrell et al., 1992). Therefore, exercise training could be incorporated within the interventions to combat CVD.
In conclusion, the fine-tuned control of circulation is of primary importance, as the cardiac output and the distribution of blood flow must be redirected to meet the ever changing metabolic demand of the different tissues and organs. CVD are a major cause of morbidity and mortality worldwide, and increase considerably the burden of health care cost. Parasympathetic and sympathetic outflow plays a key role in circulatory control and cardiovascular health. Decreased cardiac vagal control is associated with reduced HRV, poor cardiorespiratory fitness, increased risk of adverse cardiac events and insulin resistance. Exercise training brings about various health and fitness related benefits, such as improvement in HRV and cardiac electrical stability, and reduced the risk of adverse cardiac events. Therefore, exercise should be included within the interventions to improve poor cardiovascular fitness and tackle CVD.
References
ADREANI, C. M., Hill, J. M. & Kaufman, M. P. Responses of group III and IV muscle afferents to dynamic exercise. J Appl Physiol. 1997, 82, 1811-7.
ANDERSON P, SALTIN B. Maximal perfusion of skeletal muscle in man. J Physiol, 1985, 366: 233–249.
ALAM, M. SMIRK, F. H. (1937) Observations in man upon a blood pressure raising reflex arising from the voluntary muscles. J Physiol. 1937, 89, 372-83.
ANDRES, K. H., VON DURING, M. & SCHMIDT, R. F. (1985) Sensory innervation of the Achilles tendon by group III and IV afferent fibers. Anat Embryol (Berl), 172, 145-56.
BARMAN, S. M. & GEBBER, G. L. (1985) Axonal projection patterns of ventrolateral medullospinal sympathoexcitatory neurons. J Neurophysiol, 53, 1551-66.
BARMAN, S. M. & WURSTER, R. D. (1975) Visceromotor organization within descending spinal sympathetic pathways in the dog. Circ Res, 37, 209-14.
BARRON, H. V. & LESH, M. D. (1996) Autonomic nervous system and sudden cardiac death. J Am Coll Cardiol, 27, 1053-60.
BAUER, R. M., IWAMOTO, G. A. & WALDROP, T. G. (1989) Ventrolateral medullary neurons modulate pressor reflex to muscular contraction. Am J Physiol, 257, R1154-61.
BERNTSON, G. G., and CACIOPPO, J. T. (2007). “Heart rate variability: stress and psychiatric conditions,” inDynamic Electrocardiography, edsM. Malik and J. Camm(Oxford, UK: Blackwell Publishing), 57–64.
BILLMAN, G. E., SCHWARTZ, P. J. & STONE, H. L. (1982) Baroreceptor reflex control of heart rate: a predictor of sudden cardiac death. Circulation, 66, 874-80.
BILLMAN, G. E., SCHWARTZ, P. J. & STONE, H. L. (1984) The effects of daily exercise on susceptibility to sudden cardiac death. Circulation, 69, 1182-9.
BILLMAN, G. E. (2002) Aerobic exercise conditioning: a nonpharmacological antiarrhythmic intervention. J Appl Physiol, 92, 446-54.
BOLME, P. & FUXE, K. (1970) Adrenergic and cholinergic nerve terminals in skeletal muscle vessels. Acta Physiol Scand, 78, 52-9.
BLONQVIST CG, SALTIN B. Cardiovascular adaptations to physical training. Annual Review of Physiology 1983;45:169–189.
BLOMQUIST, T. M., PRIOLA, D. V. & ROMERO, A. M. (1987) Source of intrinsic innervation of canine ventricles: a functional study. Am J Physiol, 252, H638-44.
BORST, C. & KAREMAKER, J. M. (1983) Time delays in the human baroreceptor reflex. J Auton Nerv Syst, 9, 399-409.
BUCCELLETTI, E., GILARDI, E., SCAINI, E., GALIUTO, L., PERSIANI, R., BIONDI, A., et al. (2009). Heart rate variability and myocardial infarction: systematic literature review and metanalysis.Eur. Rev. Med. Pharmacol. Sci. 13, 299–307.
CALARESU, F. R., FAIERS, A. A. & MOGENSON, G. J. (1975) Central neural regulation of heart and blood vessels in mammals. Prog Neurobiol, 5, 1-35.
CIRIELLO, J., CAVERSON, M. M. & POLOSA, C. (1986) Function of the ventrolateral medulla in the control of the circulation. Brain Res, 396, 359-91.
CHEN, H. I., LEE, T. M. & CHAI, C. Y. (1972) Cardiac arrhythmias of vagal and sympathetic origin induced by carotid occlusion. Chin J Physiol, 21, 117-24.
COLE, C. R., BLACKSTONE, E. H., PASHKOW, F. J., SNADER, C. E. & LAUER, M. S. (1999) Heart-rate recovery immediately after exercise as a predictor of mortality. N Engl J Med, 341, 1351-7.
DAMPNEY, R. A. & MOON, E. A. (1980) Role of ventrolateral medulla in vasomotor response to cerebral ischemia. Am J Physiol, 239, H349-58.
DAMPNEY, R. A. (1994) Functional organization of central pathways regulating the cardiovascular system. Physiol Rev, 74, 323-64.
DE HERT S. Physiology of hemodynamic homeostasis. Best Pract Res Clin Anaesthesiol. 2012 Dec;26(4):409-19. doi: 10.1016/j.bpa.2012.10.004. PMID: 23351228.
FELDBERG, W. & GADDUM, J. H. (1934) The chemical transmitter at synapses in a sympathetic ganglion. J Physiol, 81, 305-19.
FARRELL, T. G., ODEMUYIWA, O., BASHIR, Y., CRIPPS, T. R., MALIK, M., WARD, D. E. & CAMM, A. J. (1992) Prognostic value of baroreflexsensitivity testing after acute myocardial infarction. Br Heart J, 67, 129-37
FURNIVAL, C. M., LINDEN, R. J. & SNOW, H. M. (1973a) Chronotropic and inotropic effects on the dog heart of stimulating the efferent cardiac sympathetic nerves. J Physiol, 230, 137-53.
GEBBER, G. L., ZHONG, S., ZHOU, S. Y. & BARMAN, S. M. (1997) Nonlinear dynamics of the frequency locking of baroreceptor and sympathetic rhythms. Am J Physiol, 273, R1932-45.
GILES, W. & SHIMONI, Y. (1989) Comparison of sodium-calcium exchanger and transient inward currents in single cells from rabbit ventricle. J Physiol, 417, 465-81.
GULLI, G., CEVESE, A., CAPPELLETTO, P., GASPARINI, G. & SCHENA, F. (2003) Moderate aerobic training improves autonomic cardiovascular control in older women. ClinAutonRes, 13, 196-202.
GUNN, C. G., SEVELIUS, G., PUIGGARI, J. & MYERS, F. K. (1968) Vagal cardiomotor mechanisms in the hindbrain of the dog and cat. Am J Physiol, 214, 258-62.
GUYENET, P. G. & KOSHIYA, N. (1995) Working model of the sympathetic chemoreflex in rats. Clin Exp Hypertens, 17, 167-79.
HAINSWORTH, R. (1995) Cardiovascular reflexes from ventricular and coronary receptors. Adv Exp Med Biol, 381, 157-74.
HULL, S. S., JR., EVANS, A. R., VANOLI, E., ADAMSON, P. B., STRAMBA-BADIALE, M., ALBERT, D. E., FOREMAN, R. D. & SCHWARTZ, P. J. (1990) Heart rate variability before and after myocardial infarction in conscious dogs at high and low risk of sudden death. J Am CollCardiol, 16, 978-85.
HULL, S. S., JR., VANOLI, E., ADAMSON, P. B., VERRIER, R. L., FOREMAN, R. D. & SCHWARTZ, P. J. (1994) Exercise training confers anticipatory protection from sudden death during acute myocardial ischemia. Circulation, 89, 548-52.
IELLAMO, F., LEGRAMANTE, J. M., MASSARO, M., RAIMONDI, G. & GALANTE, A. (2000) Effects of a residential exercise training on baroreflexsensitivity and heart rate variability in patients with coronary artery disease: A randomized, controlled study. Circulation, 102, 2588-92.
IRISAWA, H., BROWN, H. F. & GILES, W. (1993) Cardiac pacemaking in the sinoatrial node. Physiol Rev, 73, 197-227.
JOHNSON, T. A., GRAY, A. L., LAUENSTEIN, J. M., NEWTON, S. S. & MASSARI, V. J. (2004) Parasympathetic control of the heart. I. An interventriculo-septal ganglion is the major source of the vagal intracardiac innervation of the ventricles. J Appl Physiol, 96, 2265-72.
KIERNAN JA, RAJAKUMAR N. (2014) Barr’s the Human Nervous System: An Anatomical Viewpoint. Wolters Kluwer.
LEVY, M. N. (1971) Sympathetic-parasympathetic interactions in the heart. Circ Res, 29, 437-45.
LEVY, M. N. (1984) Cardiac sympathetic-parasympathetic interactions. Fed Proc, 43, 2598-602.
LEVY, M. N. (1997) Neural control of cardiac function. Baillieres Clin Neurol, 6, 227-44.
LEVY, M. N., MARTIN, P. J., IANO, T. & ZIESKE, H. (1970) Effects of single vagal stimuli on heart rate and atrioventricular conduction. Am J Physiol, 218, 1256-62.
LEVY, M. N. & ZIESKE, H. (1969) Autonomic control of cardiac pacemaker activity and atrioventricular transmission. J Appl Physiol, 27, 465-70.
MCALLEN, R. M. & SPYER, K. M. (1976) The location of cardiac vagal preganglionic motoneurones in the medulla of the cat. J Physiol, 258, 187-204.
MCALLEN, R. M. & SPYER, K. M. (1978) Two types of vagal preganglionic motoneurones projecting to the heart and lungs. J Physiol, 282, 353-64.
MACKAAY, A. J., OP'T HOF, T., BLEEKER, W. K., JONGSMA, H. J. & BOUMAN, L. N. (1980) Interaction of adrenaline and acetylcholine on cardiac pacemaker function. Functional inhomogeneity of the rabbit sinus node. J Pharmacol Exp Ther, 214, 417-22.
MENSAH G.A., ROTH G.A., FUSTER V. "The global burden of cardiovascular diseases and risk factors: 2020 and beyond". J Am Coll Cardiol 2019;74:2529-2532.
MOLGAARD, H., SORENSEN, K. E. & BJERREGAARD, P. (1991) Attenuated 24-h heart rate variability in apparently healthy subjects, subsequently suffering sudden cardiac death. ClinAutonRes, 1, 233-7.
MURRAY C.J.L., ARAVKIN A.Y., ZHENG P., et al. "Global burden of 87 risk factors in 204 countries and territories, 1990–2019: a systematic analysis for the Global Burden of Disease Study 2019". Lancet 2020;396:1223-1249.
ODEMUYIWA, O., MALIK, M., FARRELL, T., BASHIR, Y., POLONIECKI, J. & CAMM, J. (1991) Comparison of the predictive characteristics of heart rate variability index and left ventricular ejection fraction for all-cause mortality, arrhythmic events and sudden death after acute myocardial infarction. Am J Cardiol, 68, 434-9.
O'LEARY, D. S. (1996) Heart rate control during exercise by baroreceptors and skeletal muscle afferents. Med Sci Sports Exerc, 28, 210-7.
OSTERRIEDER, W., NOMA, A. & TRAUTWEIN, W. (1980) On the kinetics of the potassium channel activated by acetylcholine in the S-A node of the rabbit heart. Pflugers Arch, 386, 101-9.
PAGANI, M., SOMERS, V., FURLAN, R., DELL'ORTO, S., CONWAY, J., BASELLI, G., CERUTTI, S., SLEIGHT, P. & MALLIANI, A. (1988) Changes in autonomic regulation induced by physical training in mild hypertension. Hypertension, 12, 600-10.
PARATI, G., DI RIENZO, M. & MANCIA, G. (2000) How to measure baroreflexsensitivity: from the cardiovascular laboratory to daily life. J Hypertens, 18, 7-19.
PARKER T, BREALEY D, DYSON A, MERVYN SINGER M. Optimising organ perfusion in the high-risk surgical and critical care patient: a narrative review. British Journal of Anaesthesia. 2019, 123 (2): 170e176.
PETERSON D.F, ARMSTRONG R.B, and Laughlin MH. Sympathetic neural influences on muscle blood flow in rats during submaximal exercise. J Appl Physiol, 1988, 65: 434–440.
POBER, D. M., BRAUN, B. & FREEDSON, P. S. (2004) Effects of a single bout of exercise on resting heart rate variability. Med SciSports Exerc, 36, 1140-8.
POWERS SK, and HOWLEY E.T. (2012). Exercise Physiology: Theory and Application to Fitness and Performance, (8th Edition). New York, NY: McGraw Hill.
RAVEN, P. B., FADEL, P. J. & OGOH, S. Arterial baroreflex resetting during exercise: a current perspective. Exp Physiol. 2006, 91, 37-49.
RAVITS, J. M. (1997) AAEM minimonograph #48: autonomic nervous system testing. Muscle Nerve, 20, 919-37.
REIS, D. J. & CUENOD, M. (1965) Central neural regulation of carotid baroreceptor reflexes in the cat. Am J Physiol, 209, 1267-77.
ROSEMBLUETH, A. & SIMEONE, F. (1934 ) The interactions of vagal and accelerator effects on heart rate. Am J Physiol, 110, 42-55.
ROSS, C. A., RUGGIERO, D. A., PARK, D. H., JOH, T. H., SVED, A. F., FERNANDEZ-PARDAL, J., SAAVEDRA, J. M. & REIS, D. J. (1984) Tonic vasomotor control by the rostral ventrolateral medulla: effect of electrical or chemical stimulation of the area containing C1 adrenaline neurons on arterial pressure, heart rate, and plasma catecholamines and vasopressin. J Neurosci, 4, 474-94.
ROSS, J., JR. (1983) Cardiac function and myocardial contractility: a perspective. J Am Coll Cardiol, 1, 52-62.
ROWELL LB. Human Cardiovascular Control. New York: Oxford Univ. Press, 1993, p. 1–483.
SHIELDS, R. W., JR. (1993) Functional anatomy of the autonomic nervous system. J Clin Neurophysiol, 10, 2-13.
SCHWARTZ, P. J., ZAZA, A., PALA, M., LOCATI, E., BERIA, G. & ZANCHETTI, A. (1988) Baroreflex sensitivity and its evolution during the first year after myocardial infarction. J Am Coll Cardiol, 12, 629-36.
SUN, M. K. & GUYENET, P. G. (1985) GABA-mediated baroreceptor inhibition of reticulospinal neurons. Am J Physiol, 249, R672-80.
SOMERS, V. K., CONWAY, J., JOHNSTON, J. & SLEIGHT, P. (1991) Effects of endurance training on baroreflexsensitivity and blood pressure in borderline hypertension. Lancet, 337, 1363-8.
STANDISH, A., ENQUIST, L. W. & SCHWABER, J. S. (1994) Innervation of the heart and its central medullary origin defined by viral tracing. Science, 263, 232-4.
SUNAGAWA, K., KAWADA, T. & NAKAHARA, T. (1998) Dynamic nonlinear vago-sympathetic interaction in regulating heart rate. Heart Vessels, 13, 157-74.
SZENTAGOTHAI, J. (1964) The Structure of the Autonomic Interneuronal Synapse. Acta Neuroveg (Wien), 26, 338-59.
TASK FORCE OF THE EUROPEAN SOCIETY OF CARDIOLOGY AND THE NORTH AMERICAN SOCIETY OF PACING AND ELECTROPHYSIOLOGY (1996) Heart rate variability. Standards of measurement, physiological interpretation, and clinical use. . Eur Heart J, 17, 354-81.
THIMM, F. & BAUM, K. Response of chemosensitive nerve fibers of group III and IV to metabolic changes in rat muscles. Pflugers Arch. 1987 410, 143-52.
UENO, L. M., HAMADA, T. & MORITANI, T. (2002) Cardiac autonomic nervous activities and cardiorespiratory fitness in older men. J GerontolA BiolSciMed Sci, 57, M605-10.
VANHOUTTE, P. M. & LEVY, M. N. (1980) Prejunctional cholinergic modulation of adrenergic neurotransmission in the cardiovascular system. Am J Physiol, 238, H275-81.
VOS T., LIM S.S., ABBAFFATI C., et al. "Global burden of 369 diseases and injuries in 204 countries and territories, 1990–2019: a systematic analysis for the Global Burden of Disease Study 2019". Lancet 2020;396:1204-1222.
WANG, J., WANG, X., IRNATEN, M., VENKATESAN, P., EVANS, C., BAXI, S. & MENDELOWITZ, D. (2003a) Endogenous acetylcholine and nicotine activation enhances GABAergic and glycinergic inputs to cardiac vagal neurons. J Neurophysiol, 89, 2473-81.
WANG, W. Z., YUAN, W. J., YANG, J., WANG, J. W., TANG, C. S. & SU, D. F. (2003b) Involvement of I(1)-imidazoline receptors in baroreceptor reflex in the caudal ventrolateral medulla of rats. Brain Res, 960, 16-24.
WARNER, H. R. & COX, A. (1962) A mathematical model of heart rate control by sympathetic and vagus efferent information. J Appl Physiol, 17, 349-55.
WILLETTE, R. N., PUNNEN, S., KRIEGER, A. J. & SAPRU, H. N. (1984b) Interdependence of rostral and caudal ventrolateral medullary areas in the control of blood pressure. Brain Res, 321, 169-74.
Comments